Serviços Personalizados
Journal
Artigo
Indicadores
-
Citado por SciELO
-
Acessos
Links relacionados
-
Similares em SciELO
Compartilhar
Portugaliae Electrochimica Acta
versão impressa ISSN 0872-1904
Port. Electrochim. Acta vol.31 no.5 Coimbra out. 2013
https://doi.org/10.4152/pea.201305249
Nanotoxicity - an electrochemist's perspective
Christopher Batchelor-McAuley, Kristina Tschulik and Richard G. Compton*
Department of Chemistry, Physical and Theoretical Chemistry Laboratory, University of Oxford, South Parks Road, Oxford OX1 3QZ, United Kingdom
Abstract
This article highlights the fundamental role of mass-transport for interfacial reactions. First, the dissolution of particulate CaCO3 is discussed demonstrating how the dimensions of the dissolving particle can 'switch' the reaction mechanism from being diffusion to surface controlled. Second, the influence of mass-transoprt on electrochemical reactions is considered, specifically considering how electrode modification can alter the observed voltammetric response in the absence of changing the electrochemical mechanism or the rate of electron transfer. Finally, these observations on the chemically controlling role of mass-transport are concluded by considering nanoparticle toxicity and how 'size effects' may be exhibited even in the absence of altered thermodynamics or interfacial kinetics of the reactions involved.
Keywords: Nanoparticles, Mass-transport, Oxygen-reduction, Nanotoxicity.
Introduction
Some years ago one of us spent considerable time studying the kinetics and mechanism of a reaction familiar to students the world over, namely the dissolution of calcium carbonate in acidic media, [1, 2]

The reaction is of obvious high importance in natural geological systems controlling for example cave formation (see Fig. 1) and water 'hardness' but at the time it was also important because of the practice of 'lake liming' - the addition of particulate calcium carbonate to natural aquifers which had attained toxically low pH values because of the effects of 'acid rain' (Fig. 2 a) and b) lake liming in practice today c) illustrates the effects of acid rain on foliage).
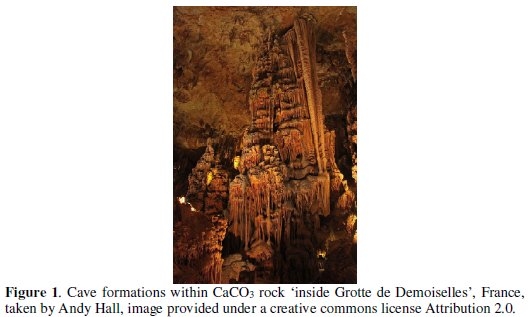
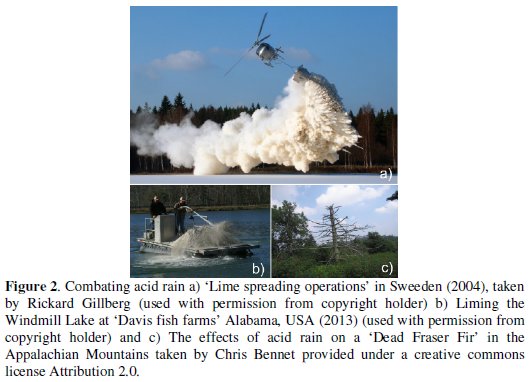
To carry out this study we invented a novel flow cell in which a 'detector electrode' was positioned downstream of a solid sample of calcite (for example semi-optical grade Icelandic spar) and used to monitor the consumption of protons from a solution as a result of contact with the CaCO3 solid. The well-defined and modelable cell hydrodynamics allowed us to measure the heterogeneous 'rate law' and rate constant for the reaction (1)

where [H+]0 signifies the proton concentration at the surface of the dissolving and reacting solid.
The flow cell experiments were carried out on macroscopic samples of solid calcium carbonate. In order to probe the interfacial kinetics we had to flow the solution very fast otherwise the solid dissolved at a rate simply controlled by the rate of mass transport to the calcite surface. Indeed previous measurements - in static solutions - had concluded that the reaction was 'diffusion controlled'. By flowing we had outrun the transport and could probe, for the first time, the interfacial kinetics of the surface reaction! We then considered the implications of our data for the dissolution of calcite particles; considering them for simplicity as reacting spheres and supposed that when added to a lake - as in the liming activity - that these would slowly descend to the bottom of the lake with a steady terminal velocity. The rate of mass-transport to the surface of a solid 'falling sphere' has been addressed by chemical engineers and is characterised by a mass-transport coefficient, [3] kMT, where

A is the species reacting on the surface and [A]bulk is its bulk concentration. Fig.3 shows how kMT varies with the particle sphere/radius.
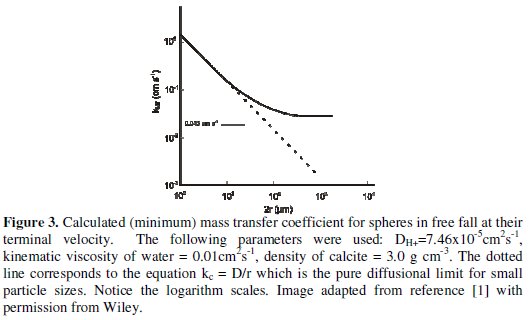
For particles of small radius, r, the transport is essentially purely diffusional and

where D is the diffusion coefficient of species A. For large radii convective transport dominates and kMT becomes radius-independent. It is interesting to compare the values of khet and kMT and consider the mechanism of proton reaction at the CaCO3 as a two step process,

where again subscript '0' denotes 'at' the calcite surface. It follows that two different rate determining steps can operate:
i) At large r when kMT < khet the slow step will be proton transport and the reaction will be controlled by the rate of proton diffusion to the surface as observed in countless experiments using macroscopic CaCO3.
ii) At low r when kMT > khet the slow step will be the surface reaction of protons with the CaCO3 surface. Fig.3 shows this will occur for r ~50 μm or less.
The kinetic data and model suggest the dissolution of small particles of CaCO3 is surface controlled in contrast to the behaviour of macroscopic size CaCO3. In short, size matters and changing the particle dimensions can alter the observed chemistry purely by altering the rate of mass transport.
Of course all electrochemists understand the role of mass-transport in their experiments. In linear sweep voltammetry the voltammetric wave appears at a potential reflecting both the prevailing interfacial kinetics and the local mass- transport. By shrinking the size of the electrode - from a macro-to a micro-(or nano-) electrode much faster interfacial processes can be observed and chemical steps 'out-run' so that a change of mechanism is perceived.
Some electrochemically irreversible processes are of high importance technologically. Thus the reduction of oxygen is crucial as a cathodic process in fuel cells. However, it is only useful if it proceeds in a four electron mechanism with the formation of water,

whereas, if it stops at the two electron stage,

the energy output of the fuel drops to a worthless level. Much effort is presently devoted to seeking efficient oxygen catalysts to reduce the overpotential for the reduction of oxygen.
The search for oxygen reduction catalysts involves making 'nanomaterials' - often some composite of exotic species such as conducting polymers, metal oxides, nanoparticle decorated carbon or nanotubes, etc., usually combined in a combinatorially complex blend! Evaluation of the 'nanocomposite' most commonly involves comparing the reduction of O2 on some substrate (say C or Au) with that of the electrode modified with a layer of the new 'electrocatalyst'. Invariably a decrease of the overpotential is seen as being manifested by a lowering of the O2 reduction linear sweep peak potential. This is deemed diagnostic of an increased rate of electron transfer. Indeed this is a possible explanation. However, this interpretation neglects the probable changes in mass- transport resulting from modifying the electrode with a nanocomposite, for a decrease in overpotential can also result from a reduced rate of mass transport as compared to the semi-infinite diffusion prevailing on the planar un-modified electrode [4, 5]. Such changes in the mass transport can easily happen from the changed geometry on the electrode surface. Thus our recent simulations show that if the nanocomposite layer has porosity or even significant roughness as compared to the flat surface deceptive shifts in the overpotential readily arise [5]. It is tempting to believe that many if not the vast majority of oxygen electrocatalysts characterised by qualitatively interpreted linear sweep voltammetry reflect nothing more than the porosity of the modifying layer rather than authentic 'catalysis' by which is meant a change of electrochemical rate constant.
It is clear that care is needed in assigning a qualitative shift of overpotential to changed electrochemical reactions if altered surface roughness or porosity occurs simultaneously with surface modification [6-8]. A further example of this type of misinterpretation - contained quite possibly in literally thousands of electroanalytical papers - concerns the modification of electrodes with layers of carbon nanotubes. In a typical experiment a planar electrode (often glassy carbon) is modified with a porous layer of nanomaterials. A vast number of compounds have been found to be oxidised or reduced at lower potentials leading to claims of 'electrocatalysis'. Whilst a variety of other possibilities for changed behaviour exist, including authentic electrocatalysis - as summarised in the admirable review of the area written by Martin Pumera [9], an obvious, simple and often unifying explanation is that the measured change reflects a shift from linear diffusion to 'thin layer' type behaviour characteristic of material trapped in nanopores within the CNT modifying layer (see Fig. 4 for a schematic showing the two types of diffusion at a modified electrode).
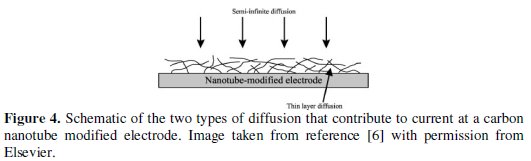
Further, the claims of improved selectivity between different analytical targets - often some subset of dopamine, serotonin, ascorbic acid, uric acid, etc. - is also readily explained by the 'porosity' model. Here the voltammetric peaks overlap under conditions of semi-infinite diffusion at a macroelectrode but shift differentially towards their various formal potentials when studied under 'thin layer' conditions as shown in simulations and confirmed experimentally [6, 10].
It is clear mass transport matters! One area where this might also illuminate controversy concerns that of nanotoxicity. A huge range of nanomaterials have recently become used in a startling variety of commercial products including TiO2 nanoparticles in the icing on doughnuts [12] or in toothpaste and sun screen; Ag nanoparticles in socks and washing machines to kill bacteria and many, many others. Fig. 5, gives a brief snapshot of the many consumer products that have been found to contain either by design or otherwise nanoparticulate material - for more information the interested reader is referred to 'The Project on Emerging Nanotechnologies' where a list of over 1600 nanoparticle containing products are provided [11].
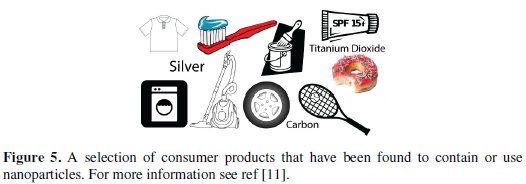
At the same time as the use of nanoparticles explodes relatively little is known about the possible toxic effect of these materials. That said silver nanoparticles represent the largest group of manmade nanoparticles reaching the environment. In the UK alone it is estimated that 8.8 tonnes of silver nanoparticles are released into the environment per annum [13]!
Some studies have appeared suggesting that nano silver is more toxic than macro-silver [14, 15]. Assuming these reports are correct, why might this be? Nanoparticles are well known to show changed behaviour from macro-scale samples of the same material. Indeed Michael Faraday (Fig. 6) showed that gold colloids have quite different optical properties to macro-gold and his work can be considered the first example of 'nanochemistry'.
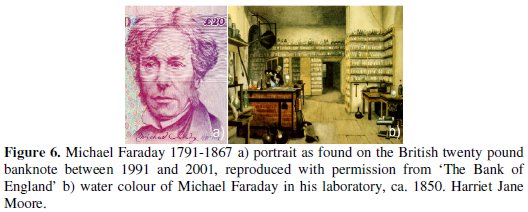
Changed behaviour at the nanoscale is commonly attributed to size effects where the reduced particle size leads to 'quantum confinement' phenomena [16]. Further, in electrochemistry the changed surface morphology - with more steps and fewer terraces as a particle shrinks in size - can profoundly alter the adsorption and hence the mechanism of many electrochemical processes. That said and noting that these two effects are predicted for nanoparticles of size tens of nanometres or smaller, is there a possible role of mass-transport?
The opinion of the authors is that mass-transport might play a significant role in nanotoxicity. We have recently been interested in the electrochemical behaviour of silver nanoparticles and studied the reduction of oxygen in neutral media on silver nanoparticle modified electrodes [17]. The process was seen to occur via the following mechanism

where kcat is the surface rate constant (cm s-1) for the solid silver catalysed decomposition of H2O2 into water and O2. The value of kcat was measured to be 0.013 cm s-1. Clearly, in light of Fig. 3, this is a value which could be slow relative to diffusion in a nanoparticle system but fast relative to transport to a macroelectrode. Indeed our experiments confirmed that at a macroelectrode reduction of oxygen to water could proceed more or less quantitatively since H2O2 formed electrochemically at the interface could decompose before it was lost to bulk solution. In contrast for isolated nanoparticles H2O2 would be lost to bulk solution because the rate of surface decomposition is slow relative to the prevailing rates of mass-transport [18].
How might the above issues impinge on the possible nanotoxicity of Ag nanoparticles? One possible mechanism is that the reduction of oxygen is coupled to oxidative dissolution of the nanoparticles forming Ag+ and encouraged by significant levels of thiols and chlorides in cellular media since both species complex strongly with Ag+:


Of course whilst Ag+ ions are in themselves toxic it is nevertheless evident that the nature of the products formed in the above scheme will be nanoparticle size dependent. For smaller particles H2O2 will be formed whilst at macro-silver H2O can be the product. Size might switch between a toxic and non-toxic product. Again mass-transport conditions can explain a size dependent phenomenon since H2O2 and other reactive oxygen species (ROS) are likely toxic in cells when formed at a concentration much higher than is ambient in cellular environment.
It is the opinion of the authors that mass transport conditions might explain the possible toxic effect of some nanoparticles and why these effects might operate for nanoparticles of sizes considerably greater than would correspond to quantum confinement effects or altered adsorption (certainly for particles greater in size than cf. tens of nanometres these latter considerations would be expected to be minimal). Indeed the mechanism applies not only to O2 reduction at silver, explaining the formation of H2O2 vs. H2O but also for any multi-step heterogeneous electron transfer process. Consider the reaction

where B is a solution phase species and khet is a surface catalysed process. Clearly, depending on the values of khet relative to prevailing mass-transport the reaction products can switch from D to B as the nanoparticle shrinks. A whole plethora of changed reactivity is possible at the nanoscale, simply because of the changed mass transport!
References
1. Compton R G, Pritchard K L, Unwin P R. Freshwater Biology, 1989;22:285288. [ Links ]
2. Compton R G, Pritchard K L, Unwin P R. J Chem Soc, Chem Comm. 1989;249-251. [ Links ]
3. Harriott P. AIChE J. 1962;8:93-101. [ Links ]
4. Gara M, Ward K R, Compton R G. Nanoscale. 2013;5:7304-7311. [ Links ]
5. Masa J, Batchelor-McAuley C, Schuhmann W, et al. Nano Res. 2013;1-8. [ Links ]
6. Streeter I, Wildgoose G G, Shao L, et al. Sensor Actuat B-Chem. 2008;133:462-466. [ Links ]
7. Kozub B R, Rees N V, Compton R G. Sensor Actuat B-Chem. 2010;143:539546. [ Links ]
8. Sims M J, Rees N V, Dickinson E J F, et al. Sensor Actuat B-Chem. 2010;144:153-158. [ Links ]
9. Pumera M. Chem Record. 2012;12:201-213. [ Links ]
10. Henstridge M C, Dickinson E J F, Aslanoglu M, et al. Sensor Actuat B-Chem. 2010;145:417-427. [ Links ]
11. Project on Emerging Nanotechnologies (2013). Consumer Products Inventory. Retrieved 29/11/2013, from http://www.nanotechproject.org/cpi. [ Links ]
12. Biello D. Nanopowder on Your Doughnuts: Should You Worry? In: Scientific American. 2013. [ Links ]
13. Whiteley C M, Valle M D, Jones K C, et al. Environ Sci: Proc Impacts. 2013;15:2050-2058. [ Links ]
14. Carlson C, Hussain S M, Schrand A M, et al. J Phys Chem B. 2008;112:13608-13619. [ Links ]
15. Cha K, Hong H-W, Choi Y-G, et al. Biotechnol Lett. 2008;30:1893-1899. [ Links ]
16. Rao C N R, Kulkarni G U, Thomas P J, et al. Chem Soc Rev. 2000;29:27-35. [ Links ]
17. Neumann C C M, Laborda E, Tschulik K, et al. Nano Res. 2013;6:511-524. [ Links ]
18. Batchelor-McAuley C, Tschulik K, Neumann C C M, et al. Submitted. 2013. [ Links ]
*Corresponding author. E-mail address: Richard.compton@chem.ox.ac.uk
Accepted 31 October 2013