Serviços Personalizados
Journal
Artigo
Indicadores
Links relacionados
Compartilhar
Portugaliae Electrochimica Acta
versão impressa ISSN 0872-1904
Port. Electrochim. Acta vol.33 no.1 Coimbra jan. 2015
https://doi.org/10.4152/pea.201501013
Comparison of Pyridazinium Electro-oxidation on Boron-doped Diamond (BDD) and SnO2 in a Basic Medium
H. Bouyaa , M. Erramia , R. Salghia,* , S. Jodehb , M. Messalic and B. Hammoutid
a Equipe de Génie de l'Environnement et de Biotechnologie, ENSA, Université Ibn Zohr, BP 1136, Agadir, Morocco
b Department of Chemistry, An-Najah National University, P. O. Box 7, Nablus, Palestine
c Chemistry Department, Faculty of Science, Taibah University, 30002, Al-Madinah Al-Mounawwara, Saudi Arabia
d LCAE-URAC18, Faculté des Sciences, Université Mohammed 1er, Oujda, Morocco
Abstract
The eco-friendly ionic liquid 1-(2-ethoxy-2-oxoethyl) pyridazinium chloride's (EOPC) newly synthesised electro-oxidation behaviors of boron-doped diamond (BDD) and SnO2 in an alkaline media were compared using galvanostatic electrolysis. Various pyridazinium and alkaline concentrations were studied. In addition, the temperature, and density current effect of pyridazinium oxidation were investigated. The BDD showed a higher activity toward pyridazinium oxidation than the SnO2. This is mainly due to the higher characteristics and the relatively inert nature of the BDD. The bulk electrolysis tests have shown that the complete removal of COD can only be achieved using a boron-doped diamond and that SnO2 only permits a partial oxidation of pyridazinium. On the basis of the chemical oxygen demand (COD) evolution released during the treatment, a total mineralization has been proposed. Finally, electrolysis has been performed in the presence of NaCl. Based on these results, it is concluded that BDD has less poisoning effects and higher activity than SnO2 for the selective electro-oxidation of pyridazinium.
Keywords: electro-oxidation, boron-doped diamond, SnO2, pyridazinium.
Introduction
Effluents from textile and paper industries contain large quantities of organic compounds and reactive dyes. These nonbiodegradable pollutants must be treated before water discharge. The commonly employed methods for color removal are adsorption [1], coagulation [2], chemical oxidation with ozone [3] and advanced oxidation processes. [4-6]. However, these processes are quite expensive and involve several operational problems. For these reasons, there has been an increasing interest in the use of new methods such as electrochemical oxidation [7-8]. Many studies have been carried out on the electrochemical treatment of organic compounds and several anode materials have been tested. However, some of them have been shown to rapidly lose efficacy due to surface fouling, while others have only selectively oxidized pollutants without their complete incineration. Complete mineralization of organics to CO2 has only been obtained using high oxygen overvoltage anodes, such as SnO2 [9-10], PbO2 [10-12] and BDD [13]. These electrodes produce hydroxyl radicals from the water discharge on their surfaces. For these kinds of electrodes, very high current efficiencies may be obtained, and higher mineralization of the organic compounds can be achieved. However, a common drawback of SnO2 and PbO2 is a short service-life [14], while BDD anodes have good chemical and electrochemical stability (even in strong aggressive media), long life, and a wide potential window for water discharge. It has been demonstrated that many biorefractory compounds such as phenols [15-16], chlorophenols [11,17,18], pesticides [19-20], and industrial wastes [21] can be completely mineralized with high current efficiency, even close to 100%, using BDD anodes. Pyridazinium was chosen as a model substrate because it was largely used in the production of azo-dyes but was biorefractory. The chemical oxidation process, such as with ozone [25], has some drawbacks including the production of large quantities of sludge or the high cost of ozone. The aim of this work was to investigate and to compare the behavior of BDD and SnO2 anodes during the bulk oxidation of pyridazinium. Furthermore, the influences of the main operating parameters, such as current density, concentration and temperature affecting pyridazinium oxidation, have been investigated for each electrode. The chemical structures of the studied pyridanium ionic liquids are given in Fig. 1.
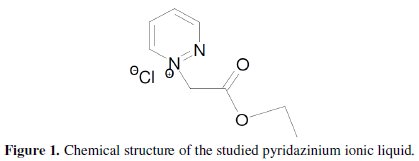
Materials and methods
Electrolytic system
Electrochemical measurements were performed using a computer controlled by a potentiostat/ galvanostat model PGZ 100 associated to the ''Volta-Master 4'' software. A conventional three-electrode cell (100 cm3) thermo regulated glass cell was used (Tacussel Standard CEC/TH). The anodes were a square plate of BDD and SnO2 electrodes with an effective surface area of 1 cm2 (per electrode), whereas the cathode was a platinum electrode, with the gap between electrodes being 0.5 cm. A saturated calomel electrode was used as a reference. Galvanostatic electrolysis was carried out with a volume of 75 cm3 of an aqueous solution of 5 ppm of pyridazinium. The range of applied current was 10-80 mA cm-2 and samples were taken at predetermined intervals during the experiment, and submitted for analysis. All tests were performed at (25 ± 3) °C in magnetically stirred and aerated solutions. In all cases, sodium chloride was added to the electrolytic cell at different concentrations. The chemical oxygen demand (COD) measurement during the processing permitted the evaluation of the kinetic of organic matter decay and the mineralization efficiency. COD was determined by the dichromate method. An appropriate amount of the sample was introduced into the prepared digestion solution (0-1500 mg O2.L-1) containing potassium dichromate, sulfuric acid and mercuric sulfate and the mixture was then incubated for 2h at 150 °C in a COD reactor (WTW CR 3200 thermoreaktor, Germany). COD concentrations were measured calorimetrically using the AFNOR method.
Results and discussion
Electrolysis on SnO2 anode
Effect of current density
Fig. 2 shows the trend of the COD/COD0 ratio and the current density during the anodic oxidation of 5 ppm of pyridazinium on the SnO2 electrode at different current densities [26-28].

At low current density (< 80 mA cm-2) the kinetic rate and COD removal were lower due to an increase in the parasitic reaction [29].
Effect of the initial pyridazinium concentration
Fig. 3 shows the effect of the initial concentration of pyridazinium on the trend of COD removal during galvanostatic electrolysis (80 mA cm-2) at 25 °C.

It can be observed that the degradation increases with the decrease of pyridazinium concentration. Furthermore, this indicated that the oxidation is under mass transport control (in respect to the theoretical model developed by Panizza & Cerisola) [13]. The variation of COD with time (see Fig. 3) showed a pseudo first-order kinetic, typical of reactions controlled by mass transport. Fig. 4 shows that ln COD0/COD increases linearly with time, confirming a mass transport process [26-29].
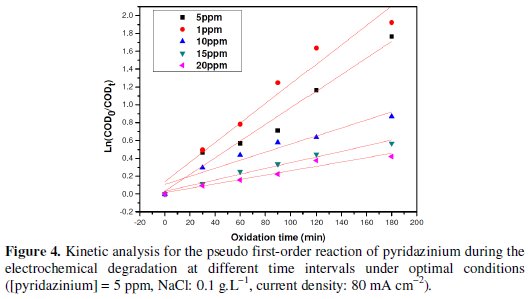
Effect of temperature
Fig. 5 shows the influence of temperature on the variation of COD removal, during galvanostatic electrolysis (80 mA cm-2).
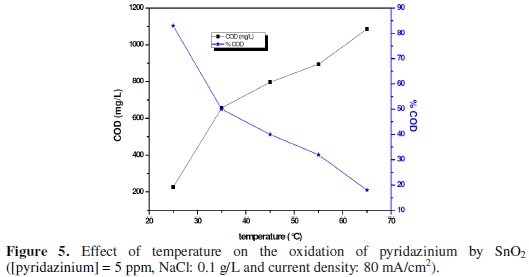
The analysis of this figure shows that COD removal increased when the temperature is close to 25 °C. However, at the temperature of 65 °C there was an anomaly during the first 60 min of electrolysis; this result can be probably explained by the oxidation of pyridazinium on the SnO2 anode, achieved at a low temperature [27].
Electrolysis on BDD anode
Effect of current density
Fig. 6 shows the trend of the COD during the oxidation of 5 ppm of pyridazinium at BDD electrode at different current densities.
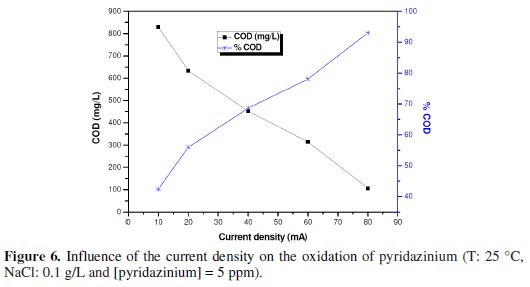
An increase of the current density resulted in an increase of the oxidation rate, similar to the result found at the SnO2 anode [26-29].
Effect of initial pyridazinium concentration
Fig. 7 shows the trend of the pyridazinium concentration during the oxidation of pyridazinium on BDD at 80 mA cm-2.
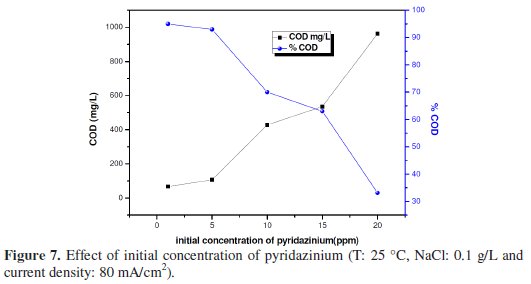
This figure reveals an evolution similar to that obtained on SnO2 when the degradation rate was more significant as the concentration was smaller [28]. In other terms, the kinetics of degradation of pyridazinium were controlled by a pseudo first-order kinetic as in the case of the SnO2 anode (Fig. 8).
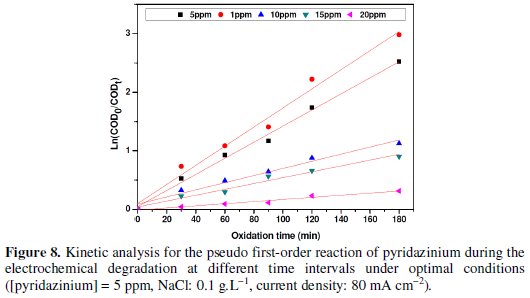
Effect of temperature
Fig. 9 shows the variation of COD according to the time of electrolysis of the pyridazinium at various temperatures.
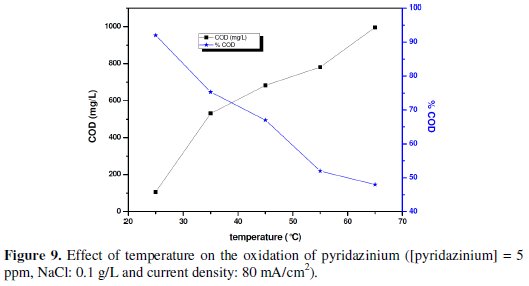
Fig. 9 also shows that the lower the temperature the better the resulting oxidation. With a temperature of 25 °C, the pyridazinium mineralization was reached at the end of 180 min around 92% while at a temperature of 65 °C, mineralization was reached at the end of 180min around 24.5%. This shows that the more the temperature was low (<65 °C) the more the oxidation of the organic pollutant was effective [29].
Fig. 2 - 9 show the comparison of the oxidation of pyridazinium using SnO2 and BDD anodes at different current densities, temperatures and different concentrations of pyridazinium. It can be observed that after 180 min, the percentage removed of COD can reach 92% on a BDD anode, whereas it was only about 83% on an SnO2 anode. This result can be related to the adsorption properties of these two materials. In fact, it is well known that the BDD anode has weak adsorption properties, compared with the SnO2 anode. Thus, hydroxyl radicals electrogenerated on the BDD anode were very weakly adsorbed and consequently more reactive towards organic oxidation reactions [22]. We can conclude that the BDD anode was the most effective electrode to degrade the pyridazinium [26].
Conclusions
In this paper, the electrochemical oxidation of pyridazinium has been studied on two different anode materials: BDD and SnO2. The results of bulk electrolysis performed under different experimental conditions have shown the following:
- Using the SnO2 and the BDD anodes, the mineralization of pyridazinium was obtained independently of the applied current density, due to the production of hydroxyl radicals on the electrode surface during electrolysis in the potential region of water oxidation.
- Pyridazinium oxidation was a diffusion-controlled process and faster COD removal was obtained at higher current densities.
- A comparison of the data obtained with the two electrode materials, each at its optimum experimental conditions, showed that faster pyridazinium mineralization and higher current efficiencies were achieved using the BDD anode.
- Pyridazinium oxidation at 25 °C significantly improved the percentage of pyridazinium elimination.
- The results of bulk electrolyses confirm the advantages of the BDD over those of the PbO2.
References
1. Bousher A, Shen X. Water Res. 1997;31:2084. [ Links ]
2. Bache D H, Hossain M D, Al-Ani S H, et al. Water Supply. 1991;9:93. [ Links ]
3. Muthukumar M, Sargunamani D, Selvakumar N, et al. Dyes Pigments. 2004;63:127. [ Links ]
4. Meric S, Kaptan D, Olmez T. Chemosphere. 2004;54:435. [ Links ]
5. Aleboyeh A, Moussa Y, Aleboyeh H. Sep Purif Technol. 2005;43:143. [ Links ]
6. Bali U, Catalkaya E, Sengul F. J Hazard Mater. 2004;114:159. [ Links ]
7. Ceron-Rivera M, Davila-Jimenez M M, Elizalde-Gonzalez M P. Chemosphere. 2004;55:1. [ Links ]
8. Fernandes A, Mora A, Magrinho A M, et al. Dyes Pigments. 2004;61:287. [ Links ]
9. Comninellis C, Pulgarin M. J Appl Electrochem. 1993;23:108. [ Links ]
10. Polcaro A M, Palmas S, Renoldi F, et al. J Appl Electrochem. 1999;29:147. [ Links ]
11. Gherardini L, Michaud P A, Panizza M, et al. J Electrochem Soc. 2001;148:D78. [ Links ]
12. Samet Y, Elaoud S C, Ammar S, et al. J Hazard Mater B. 2006;138:614. [ Links ]
13. Panizza M, Cerisola G. Electrochim Acta. 1997;51:191. [ Links ]
14. Correa-Lozano B, Comninellis C, De Battisti A. J Appl Electrochem. 1997;27:970. [ Links ]
15. Canizares P, Diaz M, Dominguez J A, et al. Ind Eng Chem Res. 2002;41:4187. [ Links ]
16. Iniesta J, Michaud P A, Panizza M, et al. Electrochim Acta. 2001;46:3573. [ Links ]
17. Boye B, Michaud P A, Marselli B, et al. New Diamond Frontier Carbon Technol. 2002;12:63. [ Links ]
18. Canizares P, Garcia-Gomez J, Rodrigo M A. J Appl Electrochem. 2003;33:917. [ Links ]
19. Brillas E, Boye B, Sires I, et al. Electrochim Acta. 2004;49:4484. [ Links ]
20. Polcaro M., Palmas M, Vacca A. Electrochim Acta. 2004;49:649. [ Links ]
21. Panizza M, Michaud P A, Cerwasola G, et al. Electrochem Commun. 2001;3:336. [ Links ]
25. Gul S, OSerindag O, Boztepe H. Turk J Chem. 1999;23:21. [ Links ]
26. Errami M, Salghi R, Abidi N, et al. Int J Electrochem Sci. 2011;6:4927. [ Links ]
27. Errami M, Salghi R, Zarrouk A, et al. Int J Electrochem Sci. 2012;7:10313. [ Links ]
28. Hachami F, Salghi R, Errami M, et al. Phys Chem News. 2010;52:107. [ Links ]
29. Bouya H, Errami M, Salghi R, et al. Int J Electrochem Sci. 2012;7:3453. [ Links ]
*Corresponding author. E-mail address: r.salghi@uiz.ac.ma
Received 1 December 2014; accepted 30 January 2015