Servicios Personalizados
Revista
Articulo
Indicadores
-
Citado por SciELO
-
Accesos
Links relacionados
-
Similares en SciELO
Compartir
Portuguese Journal of Nephrology & Hypertension
versión impresa ISSN 0872-0169
Port J Nephrol Hypert vol.29 no.2 Lisboa jun. 2015
REVIEW ARTICLE
Focus on:
II Physiological principles of acid-base balance: An integrative perspective
Princípios fisiológicos do equilíbrio ácido-base: Uma perspectiva integradora
Fernando Domingos
Department of Nephrology, Hospital Professor Doutor Fernando Fonseca. Amadora, Portugal
Instituto de Fisiologia, Faculdade de Medicina de Lisboa. Lisboa, Portugal
ABSTRACT
Normal human metabolism leads to the daily production of large amounts of volatile and non-volatile acids. The maintenance of the pH within physiological limits is a demanding task in which several mechanisms are involved. The most immediate answer comes from several physiological buffers that quickly neutralize pH deviations caused by the addition of strong acids or bases to the body. Bicarbonate/carbonic acid is the most important buffer pair of the extracellular milieu, but is chemically inefficient and depends on the continuous activity of the lung and kidney. Other physiological buffers have higher efficacy and are very important in the intracellular environment and renal tubules. The capacity of the various chemical buffers is kept by operating in an open system and by several controlling mechanisms. The lung is responsible for the elimination of the carbon dioxide (CO2) produced in the body. In metabolic disorders, respiratory adjustment of the elimination of CO2 prolongs the effect of the bicarbonate/carbonic acid buffer, but this process consumes bicarbonate. The kidney contributes to acid-base balance through several mechanisms: 1) controls the reabsorption of filtered bicarbonate; 2) regenerates bicarbonate consumed in buffer reactions; 3) eliminates non-volatile acids. Renal elimination of acid and bicarbonate regeneration is only possible due to the existence of several urinary buffers and to the ability of the kidneys to produce ammonia
Key-Words: Acid-base balance; bicarbonate; physiological buffers; renal ammonia production.
RESUMO
O metabolismo humano implica a produção diária de uma quantidade elevada de ácidos voláteis e não voláteis. A manutenção do pH dentro dos dentro de limites fisiológicos é uma tarefa exigente na qual estão envolvidos diversos mecanismos. A resposta mais imediata depende da existência de diversos tampões fisiológicos que neutralizam rapidamente desvios do pH provocados pela adição de ácidos ou bases fortes ao organismo. O par bicarbonato/ácido carbónico é o tampão mais importante do meio extracelular mas quimicamente é pouco eficaz e depende do funcionamento contínuo do pulmão e do rim. Outros tampões fisiológicos têm maior eficácia e são mais importantes no meio intracelular e no tubo renal. A capacidade dos diversos tampões químicos é mantida pelo seu funcionamento em sistema aberto e por diversos mecanismos reguladores.
O pulmão é responsável pela eliminação de CO2 produzido no organismo. Nos distúrbios metabólicos, o controlo respiratório da eliminação de ácidos voláteis prolonga o efeito do tampão bicarbonato/ácido carbónico mas este processo consome bicarbonato. O rim contribui para regulação do equilíbrio ácido-base através de diversos mecanismos: 1) controla a reabsorção de bicarbonato; 2) regenera o bicarbonato consumido nas reações tampão; 3) elimina os ácidos não voláteis. A eliminação renal de ácido e a regeneração do bicarbonato só são possíveis devido à existência de diversos tampões urinários e à capacidade do rim para gerar amónia.
Palavras-Chave: Bicarbonato; equilíbrio ácido-base; produção renal de amónia; tampões fisiológicos.
INTRODUCTION
Compared to other organic ions, such as sodium or potassium, the concentration of free hydrogen ions (H+) in biological fluids is very low, in the order of 0.00004 mmol/l (corresponding to pH 7.4). However, the appropriate [H+] in biological solute is extremely important because free H+ interfere with numerous chemical reactions, including most enzymatic systems that only work in a narrow pH range. The normal pH of the blood and extracellular fluid ranges from 7.35 to 7.45; intracellular pH is slightly lower, ranging between 6.0 and 7.4. Small deviations of [H+] disrupt critical reactions and may be incompatible with life1.
In basal conditions, the human body produces about 80 mEq/day of H+ arising from non-volatile acids2. This value excludes carbon dioxide and lactic acid that are eliminated by respiration. Non-volatile acids correspond mainly to phosphoric and sulphuric acids resultant from endogenous metabolism and food intake of proteins, and from intestinal bicarbonate loss that, physiologically, is equivalent to the addition of acid to the body3. Keeping acid-base balance is a complex task because the 80 mEq of non-volatile acids daily added to the system correspond to many thousands times the physiological [H+].
Significant variations of pH are prevented by several mechanisms, each belonging to a body line defence1,4:
1. The first line consists of a set of chemical buffers that neutralize almost immediately any changes in pH that occur when an acid or base are added to the body. Although their action is almost instantaneous, buffer systems could quickly run out if they were not replenished.
2. Breathing is the second line of defence. It adjusts CO2 removal from the body keeping pH within acceptable limits for further time. The respiratory response is not as fast as chemical buffers and it takes from several minutes to a few hours to start. Furthermore, this response is more effective in the compensation of acidosis than alkalosis because it would not be possible to retain much CO2 without impairing ventilation and blood oxygenation. Furthermore, the respiratory compensation of metabolic acid-base abnormalities is always an intermediate phase and is always partial.
3. The kidney is the third and last line of defence against pH deviations. Renal compensation may take from several hours to several days to occur but, when it does, it is the most effective response allowing simultaneously to eliminate non-volatile acid and to regenerate the bicarbonate consumed in buffer reactions during the time that elapsed until kidney response.
BUFFER SOLUTIONS
Chemically, an acid is a substance capable of releasing protons into the environment. When added to an aqueous solution, a strong acid releases H+ easily increasing free [H+]. In contrast, a weak acid dissociates more difficultly, preventing H+ from becoming free so easily. A buffer solution is one that minimizes pH changes when acids or bases are added to it. It consists of a weak acid and its corresponding conjugate anion (A-) that acts as an alkali1. A strong acid added to this solution dissociates rapidly releasing H+; however, organic anions from the buffer pair bind to free H+ ions, transiently preventing its free concentration from increasing, i.e., prevent pH from decreasing (Equation 1). After binding H+, organic anions become acids capable of releasing protons but, being weak acids, chemical buffers release protons only when the [H+] of the solution is relatively low.
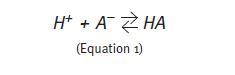
If a strong alkali (e.g.: sodium hydroxide) is added to the solution containing the buffer, the rapid dissociation of the alkali releases hydroxyl groups (OH-). In this case, the acid part of the buffer pair releases H+ and these combine with the hydroxyl groups to form water (Equation 2). In any case, the buffer intervention minimizes pH changes solution whether a strong acid or a strong alkali have been added to the solution.
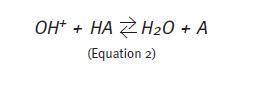
According to the Law of Mass Action, the concentration of an acid in an aqueous solution and the concentration of each of the ions in which the acid can dissociate depend on a dissociation constant (K) (Equation 3). The value of this constant is different from buffer to buffer.
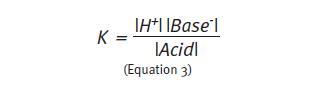
Because the value of K is a very small number, it is usually expressed in the same way as the pH, as the inverse of its logarithm (pK). Rearranging equation 3 and using the logarithmic notation, we come to the Henderson-Hasselbalch equation that allows calculating the pH in any buffer solution.
The bicarbonate/carbonic acid pair is the most important buffer of the extracellular fluid. In this buffer system, carbonic acid (H2CO3) is the weak acid and bicarbonate anion (HCO3-) is the organic base.
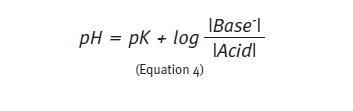
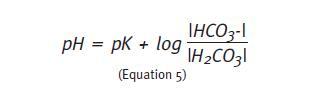
The partial pressure of CO2 (PCO2) is commonly used as a proxy for the concentration of carbonic acid because the latter dissociates very quickly into CO2 and water (H20). When the PCO2 is used to access the H2CO3/HCO3-buffer, the pK is 6.1 (Equation 6).
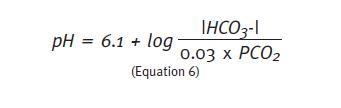
The maximum capability of any buffer system occurs when the acid and alkaline components are present in equal concentrations. In this situation, the quotient (Equation 4) equals 1, and the logarithm 1 is 0. The equation describing the HCO3-/H2CO3 buffer shows that when that happens the pH of the solution equals 6.1, i.e., the maximum efficiency of this buffer solution occurs at this pH1. The more the pH of the initial solution deviates from 6.1 the higher will be the change in pH of the final solution when an acid or alkali is added to the system. The physiological pH of the extracellular environment is approximately 7.4. Therefore, the effectiveness of bicarbonate buffer at this pH is small because most of the buffer solution is in the alkaline form (20 times more HCO3- than CO2). In case of acidosis, PCO2 has little possibility to reduce further. Indeed, the physiologic efficiency of HCO3-/ H2CO3 buffer is only possible because it works on an open system, together with other chemical buffers, and because two organs lung and kidney are continuously regulating the concentrations of CO2 and HCO3, respectively.
Other chemical buffers assist bicarbonate in maintaining the physiological pH, namely phosphate.
Phosphate exists in the body in two common forms: the acid form (H2PO4-) and the organic base (HPO42-) that has the ability to bind free H+. The pK of the phosphate buffer is 6.8, closer to the physiological pH of the body. Therefore, phosphate is a more effective buffer than bicarbonate. However, phosphate concentration in the extracellular fluid is relatively low and only contributes to approximately 8% of the buffer capacity of the extracellular compartment.
Phosphate is an important buffer in the intracellular milieu and renal tubules, where its concentration is higher and where the physiological pH is even closer to its pK1.
Proteins, mainly intracellular proteins, are important buffers responsible for 60-70% of the buffering capacity of the body. Many proteins have amphoteric properties, i.e., have groups that allow them to accept both acids and bases, which make them extremely versatile buffer systems. Furthermore, because of their abundance in various organic compartments, in particular in blood and within cells, constitute a huge buffer supply capable of neutralizing large amounts of strong acid or alkali added to the system1.
Haemoglobin (Hg) is an intracellular protein and its buffering capacity is extremely important for normal functioning of the HCO3-/H2CO3 buffer: CO2 transport in blood requires its diffusion into the interior of erythrocytes and binding H+ to Hg. This mechanism releases HCO3- and is a good example of an open system operation2. In metabolic deviations of the extracellular pH, the buffering effect of intracellular proteins is only possible due to the ability of CO2 to rapidly cross the cell membrane.
The bone is another important source of chemical buffers. A major part of the body CO2 is stored in bones as calcium carbonate and sodium carbonate; bone also has a huge reserve of bicarbonate, especially in hydration areas4. Frequently, the buffering capacity of the bone is not recalled, perhaps because acute acidosis are the most worrisome from a clinical point of view; however, bone is very important in chronic acidosis and this fact is evident by bone demineralization in chronic acidosis.
The isohydric principle states that the various buffers in a solution work together and are equally affected by acid-base imbalance. According to this principle, the evaluation of one buffer pair allows us to infer from the situation of the other buffers in the some fluid compartment. Therefore, assessment of the HCO3-/H2CO3 buffer status is sufficient for clinical investigation of acid-base balance1.
RESPIRATORY REGULATION OF ACID-BASE BALANCE
CO2 is continuously produced from tissue metabolism. Because it easily diffuses across cell membranes, it reaches the circulation very quickly, although only a small percentage is transported dissolved in blood. Most CO2 diffuses into the interior of erythrocytes where it is rapidly hydrated and converted into carbonic acid, in a reaction catalysed by carbonic anhydrase. Carbonic acid dissociates and H+ ions bind to Hg and other intracellular proteins, whereas bicarbonate leaves the erythrocytes in exchange with chloride, contributing to the buffer capacity of the plasma1.
During blood passage through the lungs, where the PCO2 is markedly low, the reverse process occurs very quickly and CO2 diffuses out of the erythrocytes to the alveolar air. In the absence of respiratory or neurological disease involving CO2 retention, it is rapidly exhaled by alveolar ventilation1.
Apart from the elimination of the CO2 produced in tissues, controlling carbon dioxide elimination is a very important mechanism to compensate, transiently, changes in pH resulting from addition of non-volatile acids and bases1. If a strong acid is added to the extracellular fluid, free H+ bind to bicarbonate, shifting the buffer pair in the direction of carbonic acid. Since H2CO3 promptly dissociates, increasing ventilation can eliminate excess CO2. This response begins within minutes by the action of chemoreceptors present in the central nervous system in close relation with bulbar centres that control respiratory activity, which are programmed to react to changes in the pH of the extracellular fluid and cerebrospinal fluid2. This mechanism is critical to keep acid-base balance in acute metabolic acid-base changes, but respiratory compensation of acidosis consumes bicarbonate and the system would not be sustainable in the long term if bicarbonate could not be regenerated.
The response to respiratory alkalosis is less effective, thought. This fact is related with the limitations of bicarbonate buffer at the physiological pH that result in much higher bicarbonate concentration when compared to carbonic acid1, and also because it would be impossible to sharply decrease ventilation not to compromise tissue oxygenation.
KIDNEY COMPENSATION
MECHANISMS
Kidney compensation of acid-base imbalance takes several hours to occur. However, the action of the kidney is essential for sustainable acid-base balance and comprises: a) reabsorption of bicarbonate lost by glomerular filtration, b) excretion of non-volatile acids and c) regeneration of bicarbonate consumed in buffer reactions. Intratubular chemical buffers and renal production of ammonia are essential for regeneration of bicarbonate and acid excretion4.
a) Reabsorption of filtrated bicarbonate
Bicarbonate is a small ion, freely filtered at the glomerulus. Its loss in urine would be critical to the life and about 99% of the filtrated HCO3- is reabsorbed by the renal tubules. The vast majority of bicarbonate reabsorption occurs early in the proximal convoluted tube (PCT) but this process has also been demonstrated, although with less intensity, in distal convoluted tube tubule (DCT) and in principal cells of the collecting duct (CD)4.
Bicarbonate cannot cross the cell membrane in the ionized form. Reabsorption of HCO3- requires proton secretion into renal tubules. In the PCT, H+ secretion occurs by exchange with sodium (Na+) at the apical side of the tubular cell. Continuous Na+ efflux out of the tubular cell is necessary at the basolateral side of the cell membrane, assured by active sodium transport by the Na+-K+-ATPase. This pump guarantees the sodium efflux required to keep the Na+ gradient between the intracellular and extracellular compartments (Fig. 1).
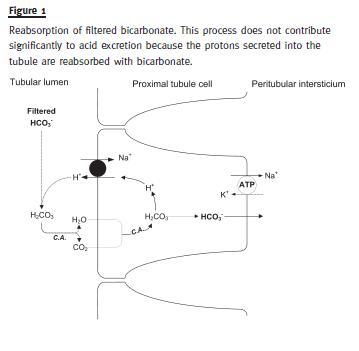
Within tubular lumen, H+ binds to filtrated HCO3- to form carbonic acid. The latter, under the action of carbonic anhydrase of the apical membrane, quickly dissociates into CO2 and water. Without electric charge, both molecules easily cross the apical membrane of the PCT cell. Once inside the cell, carbonic anhydrase catalyses the reaction that transforms CO2 and H20 into carbonic acid, which dissociates back to H+ and HCO3-. While the latter is transported across the basolateral membrane and re-enters circulation, H+ is secreted back into the tubule lumen, completing an endless cycle whose goal is to reabsorb the filtered bicarbonate4.
Reabsorption of filtered bicarbonate does not contribute to effective H+ excretion because this function implies that secreted hydrogen ions are reabsorbed across the apical membrane. Almost all protons secreted in this process came from intracellular dissociation of carbonic acid and are continuously recycled. Because there is no net excretion of H+ in this process, it does not contribute to regenerate the HCO3- consumed in buffer reactions.
The reabsorption of HCO3- suggests the existence of a maximum transport (Tm) function, i.e., it occurs at the maximum capacity until bicarbonate concentration reaches a predetermined value; above this limit, reabsorption ceases and bicarbonate is excreted in the urine5. However, unlike other biological solutes (e.g.: glucose) the Tm of bicarbonate is variable: in physiological conditions, serum [HCO3] is about 24 mEq/L and all filtered bicarbonate is reabsorbed to reach this concentration; however, if blood pH is low (e.g.: respiratory acidosis) the Tm increases to reabsorb more bicarbonate that will be used as buffer. Contrariwise, the Tm decreases in alkalosis and excess bicarbonate becomes excreted in the urine. Thus, the kidneys can tailor bicarbonate reabsorption very broadly5, having an ability to compensate acidosis or alkalosis that respiration could not reach.
b) Elimination of non-volatile acids
The regeneration of bicarbonate is the process of forming new HCO3- to replace the bicarbonate consumed in buffer reactions. It is a process independent from reabsorption of filtered bicarbonate and requires effective (net) proton excretion. Net excretion of H+ occurs mainly in the distal segments of the renal tubule, namely in the DCT and in the type A intermediate cells of the CD.
Compared to the proximal nephron, where enormous quantities of H+ need to be secreted to reabsorb filtered bicarbonate, the amount of hydrogen ions secreted in the CD is relatively small (5-10% of total). However, in the CD the H+ secretion must be carried out against a concentration gradient because urine pH was gradually lowered during its travel down the renal tubules. For this reason, in this segment of the renal tubule H+ secretion needs to be done actively by energy-consuming pumps located in apical side of the cell membrane: H+- ATPase and H+/K+-ATPase (Fig. 2)3. These pumps enable the secretion of H+ against an enormous concentration gradient, increasing the [H+] within the tubule lumen up to 900 times. This represents the concentration of free H+, corresponding to the minimum urine pH of 4.5, and the secretion into the tubule of hydrogen ions that are neutralized by several intratubular buffers.
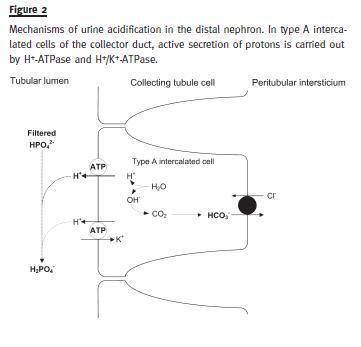
Aldosterone stimulates H+ secretion by the H+- ATPase in type A intercalated cells in the CD4. Aldosterone also stimulates H+ secretion in the principal cells of the CD by exchange with Na+ but this mechanism is quantitatively less important for net acid excretion5.
c) Bicarbonate regeneration and urinary buffers
Bicarbonate regeneration is a process closely related to renal excretion of non-volatile acid and very dependent on the existence of various urinary buffers. The latter allow retention of hydrogen ions within the renal tubule and its excretion as titratable acid or in the form of ammonia. For each H+ retained in the urine, one HCO3- ion inside the tubular cell becomes available to reenter circulation.
On average, the kidneys excrete 80 mEq of H+ per day but this value can increase to 500 mEq/day in severe acidosis. Only a tiny proportion of these hydrogen ions can be excreted in the free form3.
Since urine pH cannot decrease below 4.5 and this value corresponds to [H+] of 0.03 mEq/L, more than 2,660 litres of urine per day would be necessary to remove the normal acid production as free H+. Acid must be excreted using several intratubular buffers, namely phosphate and other less important buffers like sulphate, citrate and uric acid, or bind with ammonia4.
Phosphate is an important urinary buffer and the largest contributor to the titratable acidity of urine4.
The components of this buffer pair are HPO42- (alkaline) and H2PO4-(acid). In plasma, at pH 7.4, there is four times more alkaline than acid phosphate.
Both forms are filterable and exist in large concentrations in the urine.
Because the phosphate has a pK 6.8, close to urinary pH, the efficiency of this buffer in the urine is very high and a large part of the filtrated alkaline phosphate becomes acidic. Phosphate acidification allows the urinary excretion of 30-40 mEq of H+ per day, i.e., slightly less than half of the non-volatile acid load generated in the body under normal conditions.
Other urinary buffers, namely citrate and sulphate, will neutralize a minor portion of the hydrogen ions but approximately 50% of the daily acid load is excreted by binding to ammonia (NH3)4.
Ammonia production
Ammonia contributes to bicarbonate regeneration by two mechanisms: 1) NH3 retains hydrogen ions within distal tubule (as NH4+), allowing HCO3- present within tubular cells to be reabsorbed into the circulation; 2) ammoniagenesis in proximal tubule generates new HCO3-. The kidneys use glutamine to produce ammonia. The production occurs mainly in the cells of the PCT, although it has also been documented in thick segment of Henles loop and in the CD3. The renal catabolism of each molecule of glutamine generates one NH4+ and three new HCO3- ions, the latter being sent to the peritubular blood to regenerate bicarbonate consumed in buffer reactions (Fig. 3).
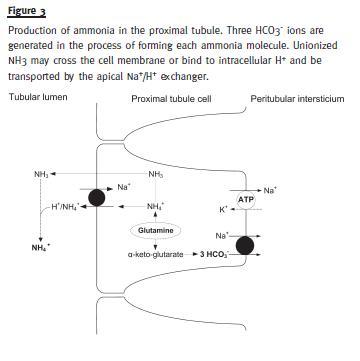
Regeneration of HCO3- by ammoniagenesis is quantitatively more important compared with regeneration reliant on urinary buffers. Furthermore, the importance of ammonia in the acid-base balance results from its production being a regulated process.
Ammonia production may increase several times if necessary. Acidosis stimulates the renal catabolism of glutamine increasing several times ammonia production allowing a larger percentage of H+ to be excreted ammonias NH4+. In alkalosis, the catabolism of glutamine decreases and is used by the liver to generate urea4.
Ammonia produced in the proximal tubule can be added to the urine as NH3, which freely diffuses through the cell membrane, or as ionized NH4+ by exchange with sodium. Either way, both forms will be reabsorbed along the renal tubule to increase the osmolality of the renal interstitium (3,5). Therefore, liquid acid excretion occurs only in distal segments of the renal tube.
Unionized ammonia freely crosses cell membranes but the CD is impermeable to ionized NH4+. Since active H+ secretion occurs in this segment of the renal tubule, when NH3 diffuses into urine binds to H+ already present in the tubular lumen and becomes trapped as NH4+, being excreted in final urine. Ammonia is not part of titratable acidity and in normal conditions it represents approximately 50% of the daily acid excretion. However, in chronic acidosis the production of ammonia increases allowing the elimination of up to 500 meq of H+ per day, becoming the most important mechanism for the compensation of acidosis4.
References
1. Hall JE. Guyton and Hall Textbook of Medical Physiology. 11th ed. Philadelphia Saunders; 2006. [ Links ]
2. Palmer BF, Narins RG, Yee J. Clinical acid-base disorders. In: Davison AM, Cameron JS, Grünfeld E, et al. editors. Oxford Textbook of Clinical Nephrology. 3rd ed. Oxford: Oxford University Press; 2005. p. 322-343. [ Links ]
3 . Alpern RJ, Hamm LL. Urinary acidification. In: DuBose TD, Hamm LL, editors. Acid-Base and electrolyte disorders A companion to Brenner & Rectors the KidneyAcid-Base and electrolyte disorders A companion to Brenner & Rectors the Kidney. Philadelphia: Saunders; 2002. p. 23-36. [ Links ]
4 . Gennari FJ, Maddox DA. Renal regulation of acid-base homeostasis: integrated response. In: Seldin DW, Giebisch G, editors. The Kidney: Physiology and Pathophysiology. 3rd ed. Philadelphia: Lippincott Williams & Wilkins; 2000. p. 2015-53. [ Links ]
5 . Lote CJ. Principles of Renal Physiology. 4th ed. Dordrecht: Kluwer Academic Publishers; 2000. [ Links ]
Professor Doutor Fernando Domingos
Nephrology Department
Hospital Professor Doutor Fernando Fonseca
Estrada IC-19
2720-276 Amadora
Conflict of interest statement: None declared
Received for publication: 5/05/2015
Accepted: 8/05/2015