Introduction
Graphical abstract
Pesticides have greatly contributed to a global increase in food productivity, profits for farmers and diseases prevention. Pesticides do not always stay in the applied location, as they are mobile in the environment, and often move through water, air and soil. Pesticides large scale application of has resulted in their remarkable persistence in the environment, representing a threat for the entire ecosystem [1]. Conventional methods employed for pesticides detection are gas chromatography and high performance liquid chromatography (HPLC) [2, 3]. Non-chromatographic methods, such as UV-Vis, infrared spectroscopy, solid-phase extraction, enzymatic techniques and mass spectrometry have also been reported for pesticides detection [4, 5]. These techniques for pesticides analysis are highly expensive, and not completely acceptable for the routine monitoring of a large number of samples. Consequently, rapid and reasonable methods of analysis are required to complement these techniques. EC methods are a key alternative to these complicated techniques, due to their extremely high sensitivity, selectivity and simplicity in experimental procedures [6-8]. Pesticides detection has been achieved through many EC techniques, such as CV [9], Differential Pulse Voltammetry (DPV) [10] and SWV [11]. SWV has the potential of monitoring pesticides through the use of GCE, by minimizing the charging current, which results in a sharp electrochemical response towards the analyte, due to an intense faradic current [12]. Over past decades, to achieve a high electrochemical response on pesticides detection, GCE surfaces have been modified by different electroactive materials viz., metal oxides [13-15], clays [16], carbonaceous nanomaterials [17-20], PNC with metal oxides [21], metals [22-23] and conducting polymers [24].
IPU is a highly selective and systematic phenyl urea herbicide, chiefly effective against innumerable annual grasses and several weeds [25]. It can easily enter in a human food chain by migration through crops in soil and water. Due to a slow degradation, its concentration goes on increasing in both ground and drinking water [26]. The maximum acceptable IPU concentration in drinking water, set by the European Union, is 0.1 g/L [27]. The continuous use of IPU over many years has resulted in the development of resistance in Phalaris Minor, causing huge losses in wheat productivity [28]. IPU use can induce oxidative stress and reduction in the chlorophyll content in wheat plants [29]. Its toxic effects have also been reported in the reproductive system of albino rats [30] and aquatic organisms, such as diatoms [31]. Some IPU cytogenetic effects have also been seen in human lymphocytes [32]. There is a vital requirement for the establishment of a fast, reliable and economical analytical technique for IPU determination.
In recent times, a lot of attention has been given to the synthesis and employment of the PNC polymer in the detection of several pesticides through EC [33-35]. It is reported in literature that IPU can be detected through HPLC [36], GC [37] and enzyme linked immunosorbent assays, using monoclonal and polyclonal antibodies [38]. The application of electrochemical luminescence sensors [39], imprinted polymer sensors [40], carbon modified electrodes [41] and sol-gel immunosorbents [42] has also been examined for low level IPU detection. Nanocomposites play different roles in nanosensors construction process, with their different compositions, morphologies and sizes. Due to the unique nanocomposites properties, they have showed significant enhancement effects on the EC determination of different analytes [43-45]. Nanocomposites have been incorporated into electrochemical sensors by the WE surface modification, and used for the investigation of various pesticides. In this study, a PMMA/M(FexOy) PNC modified GCE was developed as WE, and employed for IPU quantification, using CV and SWV EC methods. Nano M(FexOy) has been used as a very powerful determination material for biomolecules detection. Herein, PMMA/M(FexOy) PNC has been employed for the first time as a detection material for IPU quantification. Due to the M(FexOy) filler uniform dispersion and high conducting nature in PMMA, PNC/GCE exhibited good CV and SWV responses, and it is proposed as a reasonable alternative for IPU quantification. The IPU detection protocol through this work may also be used for the EC detection of different pesticides in environmental samples.
Materials and methods
Reagents
IPU, with Arelon trade name (75%, Aventis Crop Science India, Ltd.), was crystallized into ethanol, at the melting point (mp) of 157.5 ºC. Other chemicals and solvents (with a purity of >98%) were indigenously procured as analytical reagent grades, and used without further purification. All aqueous solutions were prepared with distilled water.
Ferrite Polymer Nanocomposites (FPNCs) were prepared and characterized according to their crystallite size, through X-ray Diffraction (XRD), following earlier reported procedures [46]. Methylmethacrylate (MMA) was purified through the inhibitor extraction into sodium hydroxide (NaOH: 10%, w/v), followed by distillation under reduced pressure, at the boiling point (b.p.) of 98 ºC/10 mm/Hg (mercury), d of 0.94 g/cc (cubic centimeter) and λmax (wavelength of maximum absorbance) of 2.48 nm (nanometer) [47]. PMMA/M(FexOy) PNC was prepared through MMA (2.5 × 10-3 mol/dL) 2,2ˊ-azobiisobutyronitrile (8.25 × 10-3 mol/dL) initiated polymerization, with FPNCs (0.5%, w/v), at 1200 psi (pounds per square inch), 70±1 ºC, for 6 h, in supercritical CO2 (carbon dioxide) [48]. All the electrochemical experiments were conducted at IPU concentrations ranging from 0 to 16.68 ng/dL, in 1 M HClO4, under ambient temperature. The pH of the medium during experiments was varied from 2.0 to 9.0.
Instrumentations
PNC/GCE SEM images were recorded on JEOL, JSM 6610 LV, at 0.2 KX (7 µm: micrometer) and 15 kV (kilovolt), under identical conditions, for comparable results.
AFM images were scanned under a tapping mode, over NTEGRA Prima, through ultra sharp Si cantilevers (48 N/m force constant). IPU EC studies were performed over an IVIUM potentiostat-galvanostat, using a triple electrode cell assembly.
WE fabrication
Firstly, a GCE was thoroughly finished with a fine aluminum slurry on a diamond pad, followed by ultrasonicated cleaning with acetone. A homogenous solution of coating material was prepared by heating PMMA/M(FexOy) and GO (40%) in tetrahydrofuran (1 mg/mL), at 50±1 ºC, for 20 min, followed by sonication (30 min). Then, the bare GCE top surface (3 mm diameter) was modified through its dip coating by the prepared suspension, followed by its drying at 50 ± 1 ºC, 400 mmHg, for 1 h.
Electrochemical measurements
All the EC experiments were conducted at IPU concentrations ranging from 0 to 16.68 ng/dL, in HClO4 (1 M), at pH 2 [49 - 50], under ambient temperature. GCE and PNC/GCE, a Pt foil (1 cm2) and Ag/AgCl were used as working, auxiliary and reference electrodes, respectively. The developed electrodes EC for IPU detection was conducted through CV, and the peak currents (µA) and peak potentials (V) were measured. CV was scanned under the potential range from 1.55 to -1.15 V of 0.045 V/s. IPU was quantified with SWV, by plotting a calibration graph between the maximum peak current and the respective increasing concentrations. The experimental parameters (pH of the medium, supporting electrolyte (s.e.), SWV frequency, pulse amplitude and step potential) were optimized in relation to the maximum peak current and selectivity. The SWV conditions were optimized at the intervals of 5 mV, accumulation potential of - 0.3 V, frequency of 35 Hz and amplitude of 30 mV. The LOD and LOQ were calculated from the SWV calibration curve using the formula: LOQ = 10 s/m and LOD = 3.3 s/m, where s is the intercept and m is the slope of the calibration curve.
Results and discussion
PNC/GCE surface characterization
The transfer of electrons from the coated conductive polymer matrix to the electrode is one of the key parameters in EC determination which depends on the stability and morphology of the coating material over the bare electrode. In this study, PNC/GCE surface characterization was carried out by SEM and AFM studies. SEM revealed that PMMA had a phase separated morphology, due to the tetrahydrofuran (THF) non-uniform expulsion [51]. PNC displayed a well distinct biphasic morphology corresponding to the homogeneous PMMA phase, with occasional FPNCs clusters (Fig. 1a and 1b) [52, 53].
A further insight into FPNCs qualitative dispersion into the PMMA matrix was made through AFM, which revealed its homogeneous surface texture, with an average roughness of 1.69 nm (Fig. 1c). This was associated with occasional defects, due to the solvent evaporation during casting procedures.
FPNCs dispersion into the PMMA matrix afforded PNCs with an average roughness of 7.35 nm, and an approximate particle size ranging from 17 to 400 nm (Fig. 1d). The two phase microstructure, consisting of a light matrix and relatively dark interstitial regions, indicates that PNCs interface side was heterogeneous. This accounts for the increase in PNCs average roughness over the GCE surface. The PMMA film shows that the average holes depth in PNC was 12.5 nm (Fig 1c). FPNCs reinforcement into the PMMA matrix has increased them to 25 nm (Fig. 1d).
IPU electrochemical behavior at PNC/GCE
The GCE is a key factor in the course of IPU EC, and its properties are governed by the nature of the modifying adsorbent material coating surface. In this work, PNC/GCE was found to provide good electron transfer properties and accessible electrode potential ranges, developing a highly stable WE for IPU monitoring. In PNC, the PMMA percentage in PMMA/M(FexOy) had a pronounced influence on IPU adsorption and, consequently, on its voltammetric response. Different PMMA and PMMA/M(FexOy) compositions (20%, 30% and 40% by wt.) were examined, to record the best signal response. Maximum current was attained for 40% by wt. of PMMA in PMMA/M(FexOy) PNC. There was a notable decrease in the peak current for the PMMA higher concentrations. The probable reason for that is the conducting M(FexOy) particle reduction at the modified GCE surface.
An optimized PNC coating was applied over GCE, and the working conditions were maximized to obtain the best results for IPU EC monitoring. A thorough study was carried out with a broad range of possible electrolytes, including HCl (hydrochloric acid), H2SO4 (sulfuric acid), HNO3 (nitric acid) and HClO4, to evaluate the s.e. Optimum pH and best EC results for IPU were obtained with 1 M HClO4, at pH 2, at both GCE and PNC/GCE.
IPU CV studies at PNC/GCE
CV studies were performed to verify IPU electro-activity at the WE, in 1 M HClO4, at pH 2, under ambient temperature. Fig. 2 depicts IPU CV (0.5 µM), in a potential range from 0.15 to 1.5 V, at a scan rate of 25 mV/s. The CV shape indicates a quasi-reversible redox response for the adsorbed IPU at GCE and PNC/GCE, in the used conditions. The CV shows cathodic peaks at 1.08 V, which relate to electro-reduction, and an anodic peak at 1.35 V, which corresponds to IPU electro-oxidation. It was found that the magnitude of cathodic and anodic peak currents produced for IPU at PNC/GCE was much higher than that of the bare GCE, due to the PNC good electrical con ductivity and high surface area, which are believed to enhance electrons transfer. IPU CV at PNC/GCE reflected sharp redox peaks with a cathodic peak current (Ipc) and an anodic peak current (Ipa) at about -34 µA and 80 µA, respectively (Fig. 2).
Scan rate effect
Fig. 3 shows the scan rate effect on the IPU CV response at PNC/GCE in 1 M HClO4, with a gradual increase of 15, 25, 50, 75, 100, 200, 300 and 400 mV/, respectively. The redox peak currents showed a good linear relationship with the scan rate, in the potential range from 0.2 to 1.6 V.
It was seen that the peak potential did not vary with the scan rate, and that the oxidation peak height greatly increased with higher scan rates, from 15 mV/s to 400 mV/s. However, the reduction adsorption peak height did not significantly vary with an increase in the scan rate. This may be due to the ohmic drop and greater capacitive currents. Since it was found that a scan rate of 200 mV/s was best suited for IPU scanning in other optimized parameters, it was selected for all further studies.
IPU concentration effect on CV
The higher IPU concentrations effect on CV was investigated in 1 M HClO4 (pH 2), at the developed PNC/GCE. As shown in Fig. 4, a uniform CV pattern was attained with successive increments in IPU concentrations, from 0.0 to 1.2 µM. Each subsequent IPU addition up to 1.2 µM reflected an increase in the peak current, which indicated a sensitive IPU detection by the PNC/GCE.
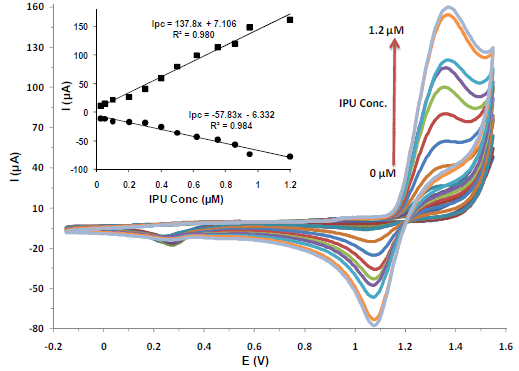
Figure 4 IPU CV on PNC/GCE in 1 M HClO4 (pH 2), with successive increases in concentration from 0.01, 0.025, 0.05, 0.075, 0.10, 0.20, 0.30, 0.40, 0.60, 0.80, 1.0 to 1.20 µM, respectively.
The CV indicated that, at higher sides, the peaks were sharp, but a response plateau appeared after IPU 1.2 µM concentration, while, at the lower end, the peaks were not significant at an IPU concentration below 0.025 µM. On the basis of these obtained results, a linear relationship was established between the peak currents and the increase in IPU concentrations.
IPU SWV at PNC/GCE
In order to check the viability of the developed PNC/GCE for IPU estimation, electrochemical parameters were optimized in SWV. The best response was obtained in the potential range from 0 to 3.0 V, with an accumulation time of 50 s, a SW frequency of 35 Hz, a SW step potential of l5 mV and an SW amplitude of 30 mV, respectively. On the successive addition of IPU into a 1 M HClO4 (pH 2) solution, a uniform increase in the peak current was found with successive higher IPU concentrations. The SWV peak currents were recorded using optimized parameters for a series of IPU concentrations ranging from 0 to 1.2 µM (Fig. 5).
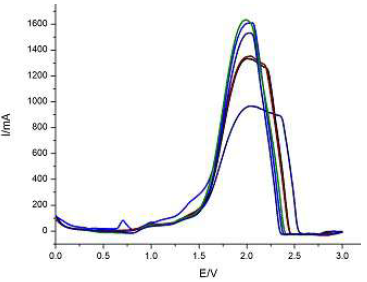
Figure 5 IPU SWV on PNC/GCE with concentrations ranging from 0.025 to 1.2 µM, in 1 M HClO4, at pH 2.
A well-defined linear calibration curve was obtained between the peak current and IPU concentration at PNC/GCE, with a LOQ of 1.98 × 10-7 M and a LOD of 6.5 × 10-8 M, respectively (Fig. 6). The SWV results specify that PNC/GCE has an excellent reproducibility for IPU estimation, which indicates that PMMA/M(FexOy) modified electrodes can be employed for electrochemical detection purposes, as an alternative source of analysis to expensive detection techniques. This makes the proposed SWV method based on PNC/GCE a fast, sensitive and better standard method. LOD and LOQ values attained by this method are significantly better than those of earlier reported methods, which indicates that SWV could be employed to analyze IPU in different environmental samples.
Interference study
Interference studies were performed to verify the applicability of PNC/GCE proposed methodology for IPU selective estimation. The existence of certain important ions in natural samples could be a probable source of interference in IPU analysis by SWV. Thus, the influence of a range of sodium, hydrochloric acid, sulfate, nitrate, magnesium, calcium, iron, zinc, potassium and copper ions (Na+, Cl-, SO4 2-, NO3 -, Mg2+, Ca2+, Fe3+, Zn2+, K+ and Cu2+, respectively) on the PNC/GCE electrochemical sensor was examined by the introduction of the ions salts in the IPU solution. The PNC/GCE tolerance limit was evaluated by increasing the interfering ions concentrations at a constant IPU concentration (5 µM). A comparison of amperometric responses showed a higher peak current value for IPU than that of other interfering ions (Fig. 7).
The outcomes revealed no significant interfering effect of the respective ions on IPU determination up to 1.4 µM. This confirms the SWV better selectivity for simultaneous IPU detection in a wide range of environmental samples.
Conclusions
Electrochemical techniques are currently the leading and most promising probes for pesticides monitoring. The prime goal of the present study was to establish a reasonable and sensitive means of IPU analysis. PMMA/M(FexOy) modified GCE (PNC/GCE), as WE, was developed, characterized and employed for IPU electrochemical detection. CV and SWV procedures for PNC/GCE provide convenient and efficient methods for IPU electrochemical estimation. CVs indicate that the current response obtained on PNC/GCE was quite better than that of the bare GCE. Therefore, the PMMA/M(FexOy) modified WE was selected for IPU monitoring. SWV studies showed IPU sensitive and selective estimation, with a LOQ of 1.98×10-7 M and a LOD of 6.5×10-8 M, without any significant interference of common coexisting ions. Additionally, a careful analysis of the results obtained from quantification limits reveals that PNC/GCE delivers an alternative method for IPU electrochemical detection. The developed protocol suggested a possibility of its application to the analytical determination of other pesticides that are usually detected at mercury electrodes with the disadvantages of being costly and more time consuming.