INTRODUCTION
The search for a healthy lifestyle, as well as the adoption of healthy habits, contribute significantly to improving the quality of life (Niven, Laird, Saunders & Phillips, 2021). In this sense, several exercise modalities arise intending to optimize physiological aspects. For example, HIIT, High-Intensity Interval Training, is characterized by brief and repeated episodes of intense activity followed by short periods of passive or active rest with low-intensity exercises (Torma et al., 2019).
Due to the possibility of adjusting certain variables, such as intensity and duration of effort and rest, active or passive rest, number and duration of sets, duration and type of recovery between sets, different HIIT protocols can be triggered (Alves, Salermo, Panissa, Franchini & Takito, 2017). These interval training models promote physiological adaptations very similar to those found in traditional aerobic exercise. Among these adjustments, some authors reported an increase in the generation of new mitochondria and modulation of oxidative enzymes, however in a much smaller period of training volume (MacInnis & Gibala, 2017; Torma et al., 2019; Silva, Galliano, & Del Vecchio, 2020).
As a high-intensity exercise, HIIT requires a high metabolic demand (Ahlert, Matzenbacher, Albarello & Halmenschlager, 2019; Souza et al., 2020), which can result in an increase in reactive oxygen species, and consequently, resulting in increased oxidative stress (OS) in various organs and tissues (Powers, Talbert, & Adhihetty, 2011). This is defined as the imbalance between the production of reactive oxygen species (ROS) and the intracellular antioxidant defense capacity (Zhang et al., 2019). Despite the studies that analyzed HITT and its effects on the body, its role on the oxidative responses induced by this modality is still scarce. Thus, this study aimed to evaluate the effects of an acute session of two HIIT protocols on oxidative parameters in rats. We hypothesize that the HW protocol will result in higher OS.
METHODS
Animals
Twenty-four male Wistar rats, with an initial age of 60 days (250 to 300 g), from the Sectorial Animal Facility of Intracellular Signaling Research Nucleus (NUPESIN) of the Universidade Federal de Sergipe (UFS) were used. The animals were divided into three groups with n= 8 each: animals that did not perform high-intensity interval exercise (SC); animals that performed high-intensity interval exercise with low work volume (LW) and animals that performed high-intensity interval exercise with high work volume between swims (HW). All experimental groups were kept in collective cages in groups of 04 rodents and under environmental conditions of temperature from 21 to 24°C and 12-hour light-dark cycle with free access to filtered water and specific food for rodents (Purina, Brazil). The procedures that were used in this study were previously approved by the Ethics Committee in Animal Research of Universidade Federal de Sergipe (CEUA/UFS), under the protocol (15/2017), and were in accordance with the Guidelines of the Brazilian College of Experiences with Animals (COBEA).
Procedures
Acute session protocol
Previously, the animals underwent an adaptation to the liquid medium, carried out for 15 days, following the protocol adapted from Contarteze, Manchado, Gobatto and Mello, (2007). In an 80 cm deep × 80 cm in diameter cylindrical tank and a water temperature of 25± 1°C with lead overload (small cotton fabric bags and Velcro) placed near the ventral region of each rodent, below the neck region distributed distally to the thoracic region, with different levels of water, load, intensity and volume.
After adaptation, the animals were submitted to two acute interval exercise protocols of high-intensity swimming, derived from the protocol by Terada et al. (2021). The LW group did a single session with 14 swimming periods lasting 20 seconds and 10-second intervals between each period, totaling a volume of 07 minutes. The HW performed a single session with 14 swimming periods lasting 35 seconds and intervals of 25 seconds between each period, totaling a volume of 14 minutes. In both HIIT cases, a load of 14% of body weight was used, with a depth of 60 cm.
Collection of biological materials
Immediately after the acute sessions, the animals were anesthetized with ketamine/xylazine (75 mg/kg + 10 mg/kg i.p) and blood (approximately 5 mL) was collected through cardiac puncture and the animals were euthanized by bleeding preceded by anesthesia. Immediately the collected blood was centrifuged at 800 g for 15 minutes at 4°C. The supernatant was then stored at -80°C. Part of the liver (1g) and gastrocnemius skeletal muscle (1 g) were removed and then washed 3 times with a 1.15% solution of KCL (Vetec, LTDA, Rio de Janeiro, Brazil), dried and weighed. After the liver and muscle were homogenized, each gram of tissue was mixed with 5 mL of KCl, 10 μL of phenylmethylsulfonyl fluoride (PMSF, 100 mmol, Sigma-Aldrich, Steinheim, Germany) and 15 μL of 10% Triton. The homogenates were then centrifuged at 3,000 g for 10 minutes at 4°C. The supernatants were stored at -80°C until further analysis of OS and tissue damage markers.
Determination of OS biomarkers
The MDA measurements were performed according to the method described by Ohkawa, Ohishi and Yagi (1979). Quantification of MDA: 100 μL of the liver and muscle tissue homogenates were incubated in Eppendorf with 350 μL of 20% acetic acid (pH 3.5) and 600 μL of thiobarbituric acid (TBA, 0.36%) for 1 hour at 85°C. Then, the Eppendorf were cooled on ice and centrifuged at 1,500 RPM for 5 minutes. Absorbance reading was performed at 532 nm. The molar extinction coefficient used was 1.54 × 105 M-1 cm-1 and the result of TBARs in nmol of MDA/g of tissue, where TBARS stands for substances reactive to thiobarbituric acid.
Determination of antioxidant capacity
Using the FRAP (Ferric Reducing Ability of Plasma) technique, a 9 μL aliquot of liver plasma was pipetted into a microplate, where 27 μL of distilled water and 270 μL of FRAP reagent were added. The plate was incubated at 37°C for 30 minutes and reading was performed at 595 nm. Ferrous sulfate (FeSO 4) was used as a standard and the results were expressed in μM of equivalents of ferrous sulfate produced.
To measure uric acid (enzymatic UV uricase-peroxidase), the commercial kit (Labtest, Santa Lagoa, Minas Gerais, Brazil) was used. Plasma (20 μL) from each animal was homogenized in specific reagents at 37± 0.2°C, and readings were taken using a spectrophotometer (Biospectrum Model SP-22 UV / Visible, Minas Gerais, Brazil) at one wavelength of 540 nm.
Statistical analysis
Data normality was tested by the Shapiro-Wilk test. Then, the Anova One Way with Bonferrini post-hoc was used to analyze the data of OS markers and antioxidant capacity. We adopted the significance level p< 0.05. All data were analyzed using the GraphPad Prism statistical program version 7.0 (GraphPad Software, San Diego, CA, USA).
RESULTS
The tissue oxidative stress lipid oxidation biomarker (Figure 1A), it shows that the protocol of single sessions of high-intensity exercise (HIIT) with low work volume (LW) was able to decrease (600.2± 89.9 vs 288.9± 15.2; -51.9%; p= 0.0001) the values of liver TBARs in rats after exercise in relation to the sedentary control group (SC). Likewise, we observed that the HIIT protocol with high workload HW also reduced (600.2± 89.9 vs 421.0± 110.7; -29.9%; p= 0.0058) the values of liver TBARs in rats after exercise compared to the sedentary control group (SC). However, when comparing the protocols, it was o observed that there was a significantly higher decrease (421.0± 110.7 vs 288.9± 15.2; 22%; p= 0.0435) in the LW protocol compared to the HW.
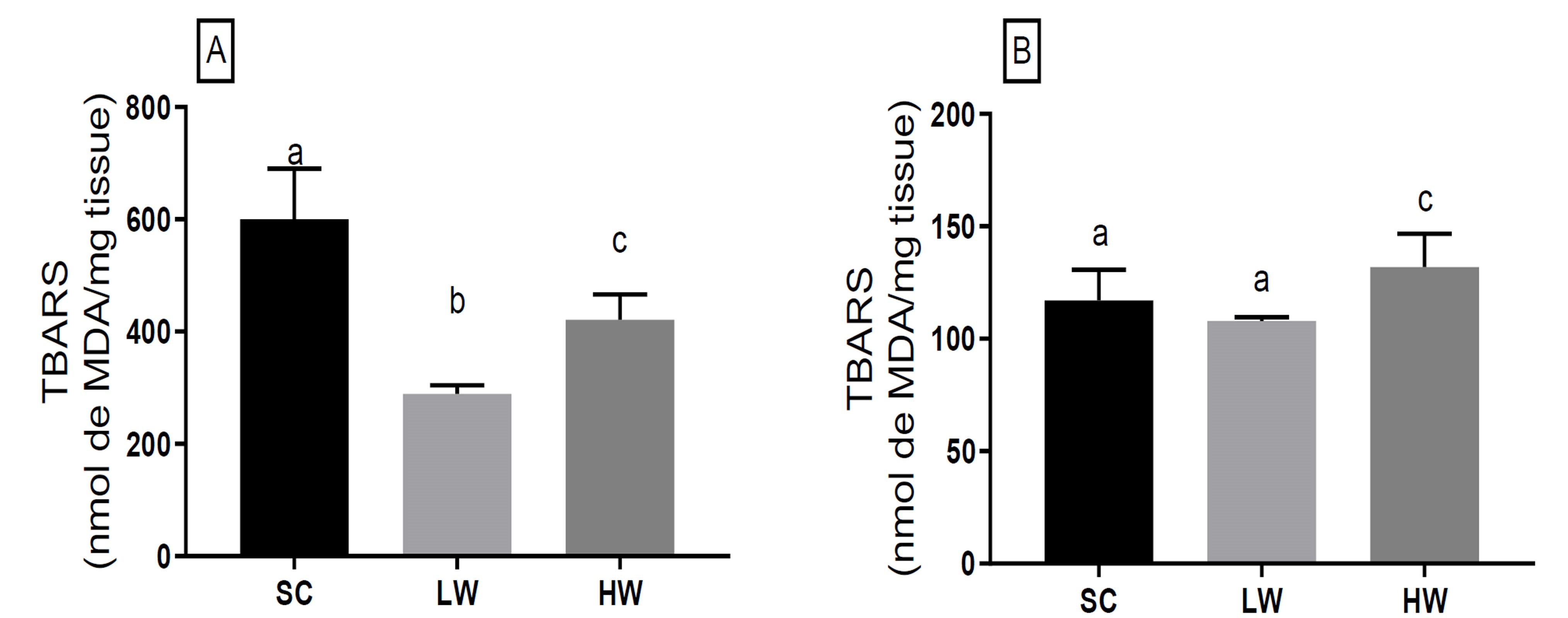
SC: sedentary control group; LW: animals that exercised with a low workload — 7 min; HW: animals that exercised with a high workload — 14 min; *values are presented as mean± standard deviation of the mean and expressed in nmol MDA/mg tissue. Samples were collected immediately after the last swimming period of the exercise session. Different letters in the figure represent a statistically significant difference at p< 0.05 between groups.
Figure 1 Effect of the high-intensity interval exercise session on oxidative stress biomarker on lipid damage in (A) liver tissue and (B) gastrocnemic skeletal muscle of rats*.
In the skeletal muscle tissue (Figure 1B), the LW protocol did not change tissue TBAR levels compared to the SC group (117.1± 13.7 vs 107.9± 5.8; -7.9%; p= 0.21). On the contrary, the HW protocol generated an increase in TBARs (117.1± 13.7 vs 131.8± 4.0; 12.6%; p= 0.012) in the tissue compared to the SC group. As seen above, the effect between protocols was 22.2% greater in HW in increasing muscle tissue TBARs than in LW.
Regarding the antioxidant capacity of the rat liver tissue measured using the iron reduction method (FRAP) (Figure 2A), suffered a strong alteration from the applied HIIT. We identified that the LW and HW protocols reduced the FRAP levels (1,210± 320.1 vs 263.2± 109.6; -78.3% and 1,210± 320.1 vs 299.4± 64.0; -75.3%; p= 0.0002) when compared to the SC group, respectively. On the other hand, we did not observe any difference in the tissue´s antioxidant capacity between LW and HW groups (263.2± 109.6 vs 299.4± 64.0; p> 0.9999), suggesting that, regardless of the protocol, HIIT causes depletion in the liver tissue’s antioxidant stores.
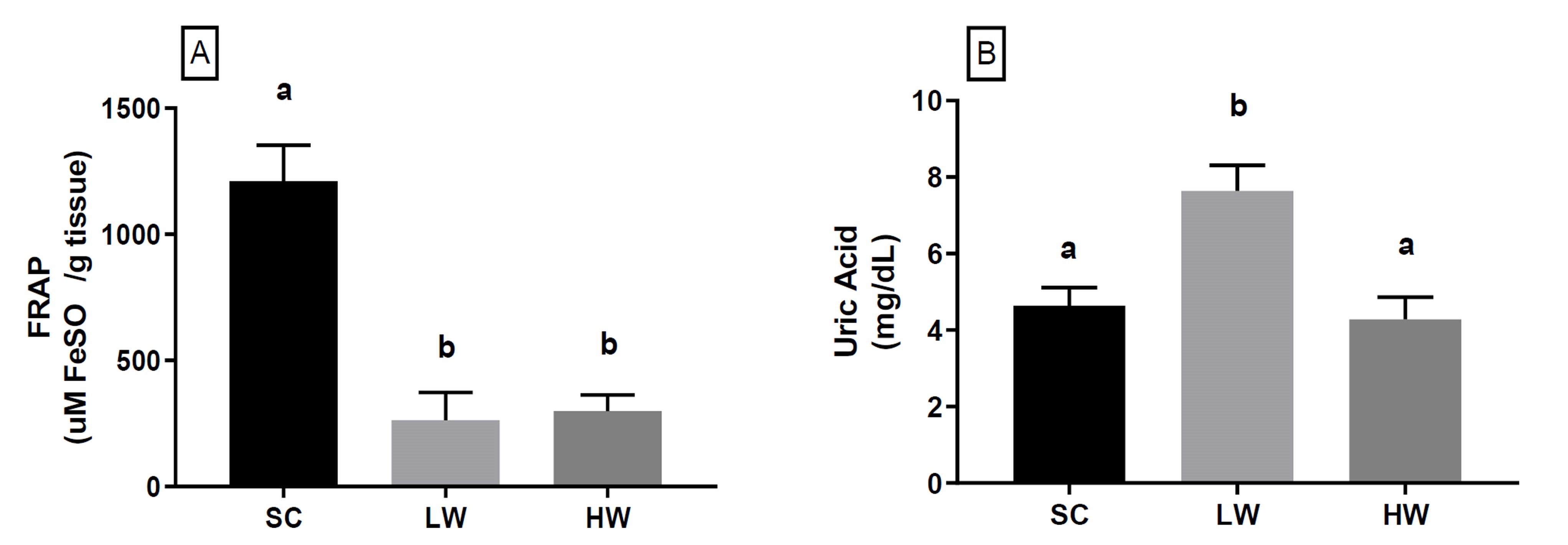
SC: sedentary control group; LW: animals that exercised with a low workload — 7 min; HW: animals that exercised with a high workload — 14 min. Values are presented as mean± standard deviation of the mean FRAP expressed in (A) μmol FeSO4/g tissue and (B) Uric acid mg/dL. Samples were collected immediately after the last swimming period of the exercise session. Different letters in the Figure represent a statistically significant difference at p< 0.05 between groups.
Figure 2 (A) Hepatic and (B) blood antioxidant capacity of rats after a high-intensity interval exercise session*.
When we analyzed the antioxidant marker uric acid (UA) in blood plasma (Figure 2B), we identified that HIIT in the LW group promoted a significant increase in the UA blood concentration compared to the SC group (4.6± 1.1 vs 7.6± 1.5; 64, 65%; p= 0.01). On the other hand, UA concentrations in the HW group were similar to SC (4.6± 1.1 vs 4.3± 0.6; p> 0.9999). But when the effects between protocols were compared, the LW group had a significant increase in blood UA compared to the HW (44%; p= 0.0046).
DISCUSSION
The present study evaluated the acute effects of two high-intensity interval exercise protocols on markers of OS in rats. Based on the results, it can be inferred that a single session of high volume HIIT promoted oxidative damage related to lipid peroxidation in the liver of Wistar rats. In addition, both high and low volume protocols reduced the antioxidant activity marker in plasma FRAP. MDA is the final product of lipid peroxidation, being used as a marker for measuring OS (Kawamura & Muraoka, 2018).
Oxidative damage to membrane lipids occurs due to an increased production of free radicals, such as reactive oxygen species (ROS) (Valko et al., 2007). During muscle contraction, ROS are produced mainly in the mitochondria, and by the activity of the enzyme xanthine oxidase and nicotinamide adenine dinucleotide phosphate (Nemes et al., 2018), and have regulatory functions in a variety of intracellular processes such as proliferation, differentiation, immune response, signaling, energy metabolism, gene expression and exercise adaptation (Schieber & Chandel, 2014; Morales-Alamo & Calbet, 2016).
However, the exacerbated increase in its production is associated with deleterious effects related to oxidative stress such as overtraining (Lewis et al., 2018). This dichotomy between the physiological effects caused by ROS and consequent oxidative stress seems to be associated with the amount of reactive oxygen and nitrogen species that is produced by intracellular processes, which may be a dose-dependent effect on the stimulus that causes it (Nikolaidis & Margaritelis, 2018).
In the present study, a decrease in the concentration of MDA was observed in the exercised groups compared to the control group, demonstrating that an acute session of HIIT did not provide significant oxidative damage, similarly to the study carried out by Bloomer and Goldfarb (2004). In addition, Ramos-Filho et al. (2015). Using the same exercise protocol, showed no significant difference immediately after exercise in the concentration of MDA in the gastrocnemius muscle of rats. The gastrocnemius muscle is formed by different types of muscle fibers, both of slow and fast contraction, which are arranged in different ways along the muscle (Ramos-Filho et al., 2015). It is known that high-intensity interval exercise requires greater activity on the fast-twitch type II contraction fibers (Holloway, Bloemberg, Silva, Quadrilatero & Spriet, 2015).
During swimming using the HIIT protocol, the gastrocnemius muscle is less recruited when compared to the tibialis anterior muscle, which contains a higher percentage of fast-twitch fibers (Nikolovski, Faulkner, Palmer & Fournier, 1996). Likewise, the less active muscle may represent a decrease in the oxidation of energy substrates during physical exercise (Ørtenblad, Westerblad, & Nielsen, 2013; Gejl et al., 2017). Therefore, it can be hypothesized that, in the models evaluated in this study, there may have been less activity of energy pathways and intracellular mechanisms responsible to produce reactive species. Therefore, it was unable to generate oxidative damage in the gastrocnemius muscle, due to the characteristics of the composition of its fibers.
Confirming this hypothesis, Ramos-Filho et al. (2015), found that the tibialis anterior muscle produces hydrogen peroxide (H2O2) in greater amounts, compared to the gastrocnemius, using the same HIIT protocol presented in our study. In more recent findings, researchers observed that the production of H2O2 depends on the characteristics of the exercise performed. HIIT was not able to increase the generation of H2O2 through mitochondrial complexes I and II in the gastrocnemius of rats, while continuous exercise presented a different scenario (Martins et al., 2018).
Adaptations to the HIIT training protocol are shown to be specific in some tissues such as skeletal muscle when compared to the continuous exercise protocol, which demonstrates that the oxidative response may vary depending on the tissue analyzed (Groussard et al., 2019). Thus, our results did not show a difference in the oxidative response in different tissues such as skeletal muscle and liver. In another tissue territory, the liver is the organ responsible for the control of glycemic homeostasis during physical exercise Gonzalez, Fuchs, Betts and Van Loon (2016), when liver cells mobilize their glycogen stores, thus providing the energy substrate needed to perform the muscle contraction (Trefts, Williams, & Wasserman, 2015). The liver is the organ responsible for the synthesis of antioxidant molecules, the main one being glutathione (Kalinina, Chernov, & Novichkova, 2014). The HIIT training protocol demonstrates an increase in the concentrations of antioxidant enzymes produced by the liver (Delwing-de Lima et al., 2018). This adaptation to training occurs due to the attack of ROS during physical exercise sessions (Powers et al., 2011).
A recent study investigated the effect of a single session of HIIT In rats, using a treadmill, on oxidative damage Melo et al. (2019), showing no significant difference in post-exercise MDA levels, although it showed improvement in antioxidant capacity in 24 h. However, due to the lack of data on exercise induced ROS quantification, it is not possible to determine whether there is a threshold of their production that results in oxidative damage, as well as the period in which the attack on biomolecules occurs, as our study portrays the moment right after the end of the exercise. Pimenta et al. (2015), observed that swimming using HIIT as a training protocol is able to improve the antioxidant defense in ovariectomized rats and hyperlipidemic diets, demonstrating the beneficial effect of reducing MDA concentrations in the gastrocnemius.